Abstract
An overview of the studies of structural characteristics of nanomaterials and nanosystems, performed by neutron scattering methods, is presented. The considered examples include optically active oxide materials, biological systems and solutions for track membrane etching. It is shown that features of structural organization at the nanoscale, including cluster formation, aggregation and packing modification, play an important role in the formation of the physical properties of materials.
Export citation and abstract BibTeX RIS

Content from this work may be used under the terms of the Creative Commons Attribution-NonCommercial-ShareAlike 3.0 licence. Any further distribution of this work must maintain attribution to the author(s) and the title of the work, journal citation and DOI.
1. Introduction
A search for novel materials demonstrating challenging physical properties and phenomena, which offer potential for the development of new technologies, is one of primary topics in modern multidisciplinary scientific research in the fields of materials science, condensed matter physics, chemistry, biophysics, medicine, etc. Significant attention in recent years has been given to nanosystems and nanostructured materials [1, 2]. The word 'νανoζ' translated from Greek as 'dwarf' describes the characteristic size of these systems or their parts, having an order of 1–100 nm. It appears that the physical properties of nanosystems and nanostructured materials can be markedly different from those in bulk, opening up new prospects for scientific research and the development of nanotechnologies.
In order to reveal the key features of the formation of physical properties of nanosystems and nanostructured materials, one needs to apply research methods that allow one to obtain information at the nanoscopic level. Neutron scattering methods—diffraction, small angle scattering, reflectometry, inelastic scattering—offer a wide range opportunities for research in the field of nanosystems and nanostructured materials. Neutrons have a number of specific properties, distinguishing them from other elementary particles: absence of electric charge, presence of magnetic moment, different scattering amplitude for different isotopes of one atom, large penetrating depth and energy comparable to that of dynamic lattice excitations [3]. These features make neutron scattering especially invaluable for studies of structural characteristics and dynamics of magnetic materials, including layered heterostructures, materials containing light atoms (H, O and Li), isotopes of the same atoms, biological objects and polymers [4–16]. Neutron investigations are of great importance for developing modern nanotechnologies in the fields of electronics, power engineering, pharmacology, medicine, chemistry and many other areas relating to the synthesis of novel materials with specified properties, which hold promise in industrial applications, the research of properties of biological molecules and membranes, polymers and the creation of new medicines.
Here we review selected results of neutron scattering studies of nanostructured materials and nanosystems, recently performed at the Frank Laboratory of Neutron Physics (Joint Institute for Nuclear Research, Dubna, Russia) [17].
2. Nanostructured materials for optoelectronic applications
At the present time, one of the topical problems in optoelectronics is a search for nanosystems, which under excitation with light could efficiently radiate energy in a given spectrum range with minimal losses [18]. The promising materials are nanostructured oxide systems synthesized by colloidal chemical methods and containing rare-earth and noble metal ions [19–21]. These materials demonstrate high stability, and controllable tuning of spectral and luminescent characteristics by variation of chemical content, and they can be easily integrated into components of optical devices. Recently, considerable attention [22] has been paid to GeO 2–Eu 2 O 3–Ag thin film and xerogel systems. A significant increase in photoluminescence intensity of E u 3+ ions in these systems with the introduction of Ag atoms has been revealed after annealing at temperatures of 600 <T O <800 °C and exciting in the ultraviolet spectrum region (figure 1).
Figure 1 Spectra of luminescence excitation of Eu 3+ ions in xerogels 95.0GeO 2–5Eu 2 O 3 (1) and 94.9GeO 2–5Eu 2 O 3–0.1Ag (2).
In order to clarify the structural mechanisms responsible for the significant modification of optical properties, small-angle neutron scattering experiments (SANS) and complementary x-ray diffraction experiments were performed on xerogels with compositions of 95GeO 2–5Eu 2 O 3, 94.9GeO 2–5Eu 2 O 3–0.1Ag and 99.9GeO 2–0.1Ag, annealed in air up to T O =850 °C [23]. It was found that the 99.9GeO 2–0.1Ag system annealed at T O =150 °C is amorphous and at T O >200 °C is crystallized into a hexagonal phase with P3221 symmetry. The 95GeO 2–5Eu 2 O 3 and 94.9GeO 2–5Eu 2 O 3–0.1Ag systems obtained at T O =150–800 °C also have a hexagonal structure with P3221 symmetry.
The experimentally measured SANS data demonstrated qualitative changes for systems 95GeO 2–5Eu 2 O 3 and 94.9GeO 2–5Eu 2 O 3–0.1Ag with an increase in annealing temperature T O from 150 to 550 °C, related to the formation of intermediate size polydisperse clusters (figure 2).
Figure 2 Experimental small angle neutron scattering curves measured for xerogels 99.9GeO 2–0.1Ag, 95.0GeO 2–5Eu 2 O 3 and 94.9GeO 2–5Eu 2 O 3–0.1Ag annealed at temperatures T O =150 (1), 550 (3) and 850 °C (2). For T O =550 °C, calculated curves are also presented.
For experimental data treatment, a model involving scattering from large objects and intermediate size clusters in an isotropic approach was used [24]:
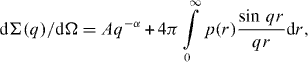
where p(r) is the neutron scattering length density for intermediate size clusters, q—scattering vector.
It was found that silver doping results in an approximately twofold decrease in the average size of intermediate clusters from 6.2 to 3.6 nm and also about twice the decrease in size distribution dispersion (figure 3). These facts can be explained by the breakage of clusters because of the formation of Eu–O–Ag chemical bonds. An increase in the relative intensity of luminescence excitation lines 7 F 0→5 L 6(λ≈395 nm) and 7 F 0→5 H 6(λ≈318 nm) of Eu 3+ ions indirectly confirms this.
Figure 3 Intermediate cluster size distribution functions for 94.9GeO 2–5Eu 2 O 3–0.1Ag and 95.0GeO 2–5Eu 2 O 3 systems annealed at T O =550 °C.
At a high annealing temperature T o =850 °C, the dissociation of the formed clusters was observed, which may be connected to the formation of a new phase of europium germanate.
Another type of prospective material for optical and quantum electronics are silicon glasses with doped oxides of the transition and rare earth elements [25]. Such glasses are characterized by high ultraviolet radiation protection and thermal stability, and they are suitable for numerous technological applications, including the development of novel modifications of laser glasses, light filters and the imitation of gem production. The glasses doped by cerium and titanium oxides are yellow–orange and the optical absorption edge can be shifted significantly by varying the CeO 2/TiO 2 concentrations ratio (figure 4) [26].
Figure 4 Optical absorption spectra of silicon glasses containing different ratios of CeO 2/TiO 2 oxides concentrations.
It was assumed that the thermal stability of the yellow–orange color in silicon glasses is a result of the formation of complex clusters Ce–Ti–O, like those formed in solid solutions [27]. However, the formation mechanisms, structural features and morphology of these clusters remain unclear. In order to study the structural characteristics of silicon glasses containing CeO 2 and TiO 2 oxides with different concentration ratios, small-angle neutron scattering experiments were performed.
The scattering curves for pure silicon glass and glasses with a molar concentration ratio of CeO 2/TiO 2 oxides 2.0/6.5 and 2.0/10.0 are shown in figure 5. The curve for the pure glass indicates scattering from large objects and can be approximated by the power law

Figure 5 SANS curves from pure silicon glass (1), and glasses with relative molar concentration CeO 2/TiO 2 2.0/6.5 (2) and 2.0/10.0 (3). The solid lines are calculated profiles.
The estimated value of the slope coefficient for pure glass is α=2.99(6). This may correspond to the scattering of microscopic air bubbles, which appeared in the manufacture of glass material. The presence of air bubbles in the glass matrix was previously observed by x-ray tomography.
The SANS curves of silicon oxides doped with CeO 2/TiO 2 exhibit somewhat different behavior. This corresponds to scattering from two different types of object, one related to larger air bubbles in the glass matrix and the other to smaller aggregates formed by Ce, Ti and O atoms. In order to estimate the characteristic value of the gyration radii (R g ) of the aggregates, assuming their isotropic monodisperse character, the experimental data were fitted by a function [28]

The comparable values R g =34.0(5) and 35.0(5) nm were obtained for glasses with CeO 2/TiO 2 concentration ratio 2.0/6.5 and 2.0/10.0, respectively, indicating weak dependence of the aggregate size on titanium oxide in the concentration range 6.5–10. The value of coefficient α, describing scattering from the glass matrix, was found to be about the same for these systems and is considerably smaller in comparison with pure glass, α=1.1(1).
3. Structural organization of mitochondria
The mitochondrion is known as the powerhouse of the cell as it produces the energy that is needed to carry on all cellular processes [29]. The intact mitochondrion (figure 6) is a complex object. For this reason, the functional and structural features of mitochondrial membranes can be studied using simpler model objects—submitochondrial particles (SMP)—which can be prepared by ultrasonication. SMP obtained by this method represents vesicles of about 40 nm in diameter formed by the mitochondrial inner membrane. Submitochondrial particles have ATP-synthase and all enzymes of the respiratory chain. Samples of SMP have significant enzymatic activity and are stable in experimental conditions.
Figure 6 Schematic representation of a mitochondrion.
In order to determine some structural parameters of SMP membranes (such as thickness and lipid–protein volume ratios), small angle scattering experiments were performed using contrast variation with samples placed into solvents with different ratios of D 2 O/(H 2 O+D 2 O) [30].
The SANS curves for submitochondrial particles in media containing 95% and 55% D 2 O are presented in figure 7. The inset of figure 7 illustrates the Kratky–Porod plot for these samples. The presence of a linear region displays the lamellar structure of the investigated object. By means of the Kratky–Porod approximation [31],
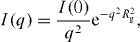
the value of I(0) was determined for each kind of solvent in the range 0.02 Å−1<q<0.08 Å−1.
Figure 7 SANS curves of SMP in media with 95% D 2 O (upper curve) and 55% D 2 O (lower curve). The Kratky–Porod plot for these samples is given in the inset.
It was found that the membrane of submitochondrial particles is heterogeneous and is formed by a lipid bilayer including proteins. The radius of gyration of SMP membrane is R g ≈15.4(2) Å. The membrane is asymmetric and the distance between the geometrical center of the membrane and the centre of mass of distribution of the inhomogeneities is about 13.3(7) Å. The estimated effective average membrane thickness is equal to 53.4(7) Å.
Recently it was shown that the effects of osmotic swelling lead to qualitative rearrangement of the ultrastructure of the mitochondrial membrane, accompanied by the formation of tightly constricted folds of the inner membrane (the so-called dried cristae) [32]. Such structural modifications are followed by changes in the main functions of the mitochondria, such as the rate of the respiratory-chain activity and the mechanism of coupling electron transport to phosphorylation [32, 33].
Since electron microscopy does not always provide unambiguous results due to the dependence of the packing of the cristae on the conditions of material fixation, detailed studies of structural re-arrangements in the mitochondrial membrane require the use of complementary methods, especially SANS. Experiments were performed with rat heart mitochondria, in which the packing of the inner membrane is qualitatively different from that observed in liver mitochondria [34]. This membrane tightly fills the total volume of mitochondria enclosed by the outer membrane.
The SANS curves for rat heart mitochondria placed in isotonic (250 mOsm) and hypotonic (90 mOsm) media are shown in figure 8. They have a contribution from a monotonic component, which can be approximated by a function B=aq−c +b and additional peaks.
Figure 8 SANS curves for mitochondria in the (1) isotonic (250 mOsm) and (2) hypotonic (90 mOsm) media. The contributions from the monotonic component are shown by the solid curves.
After extraction of the monotonic component from the experimental data, the additional contribution to SANS curves from the superimposed peaks (1, 2) was analyzed using the Gaussian function for their treatment (figure 9). For the isotonic medium, the positions of the maxima of the two peaks are related as 1:1.9(1), which correspond to first- and second-order maxima in the case of the lamellar packing of the cristae.
Figure 9 Peaks in the small-angle neutron scattering curves for heart mitochondria placed in the (a) isotonic (250 mOsm) and (b) hypotonic (90 mOsm) media. The contribution from the monotonic component B=aq−c +b was subtracted for clarity.
In this case, the experimentally determined distance d(d=2π/q) between the centers of the cristae membranes is 217(22) Å. In the hypotonic medium, the positions of the peak maxima are related by the ratio 1:1.6(1). This provides some evidence that, under hypotonic conditions, the nonlamellar membrane structures (hexagonal or cubic) are formed in mitochondria with estimated structural parameters of about 200–300 Å (figure 10) [35].
Figure 10 Schematic representation of the structural re-arrangement in mitochondrial membrane under hypotonic conditions.
In the ideal case of nonlamellar packing, the spacing ratio of is expected. The available electron microscopy data support the formation of the hexagonal phase under conditions of mitochondrial osmotic swelling. For instance, the transition of small parts of the heart mitochondrial membrane to the hexagonal packing was observed at slight swelling under anoxic conditions [36, 37]. These data clearly indicate that a larger part rather than small regions of the membrane transforms into the hexagonal phase upon osmotic swelling of the mitochondria.
Studies of the functional activity of mitochondria performed before and after irradiation with neutrons demonstrated that, under the experimental conditions used, mitochondria primarily retain their functional activity, including the ability to maintain the inner-membrane potential [38].
4. Surfactant aggregation in solutions for track membrane etching
Track membranes (TMs) are unique artificial materials that are characterized by highly uniform porosity, mechanical strength, chemical resistance and possibility variation of pore diameters and surface densities in an extended range. Due to these features, TMs have numerous scientific and technological applications as model objects containing pores with preset parameters: for instance, in industrial processes like purification, separation and concentration [39].
The important stage in the preparation of TMs is track etching by chemical treatment of ion-irradiated polymers. The shape of formed track pores is controlled by two key factors: the rate of etching latent tracks of irradiating particles and the rate of etching an unirradiated polymeric material. Depending on the ratio between these rates, the pores due to etching may be of different shapes, such as cylinders, cones and sand glasses. In addition, the pore shape is affected by the presence of surfactants in an etching solution, which are added to the initial solutions to improve wetting and to increase the uniformity of the treatment. This effect, illustrated in figure 11, can be successfully applied to control the shape of pores in track membranes, to produce high performance ultra- and microfiltration membranes [40–42], and to develop nanoporous systems with other promising characteristics and applications [43, 44]. The electron micrographs of the fracture of a track membrane fabricated by track etching in the absence of surfactants and in the presence of surfactants are shown in figure 11.
Figure 11 Electron micrographs illustrating the pore shapes in TMs obtained by the etching under different conditions of poly(ethylene terephthalate) films irradiated by a parallel beam of accelerated krypton ions: (a) etching with 3 M NaOH, 80 °C, DSBS-Na, (b) 6 M NaOH, 70 °C, DBA-Na and (c) 2 M NaOH at 80 °C in the absence of surfactants. The film thickness is 5 μm.
For the TMs fabricated by track etching in the absence of surfactants, the channels demonstrate an ordinary cylindrical geometry. The TMs resulting from etching in an alkali solution (6 mol l −1) containing sodium dodecylsulfophenoxybenzene sulfonate (DSBS-Na) show cigar-shaped pores. A decrease in the alkali concentration leads to the formation of channels with less pronounced variations in the profile along the pore length (figure 11), which can be explained by variation in the diffusion fluxes of surfactant and alkali molecules into the pores.
Although the qualitative models of pore formation (figure 12) in surfactant-containing etchants have been worked out [45, 46], many details of this process still remain unclear.
Figure 12 Illustration of the pore formation mechanism in an ion-irradiated polymer film etched with a surfactant-containing etching solution: (a) with no regard to micelles and (b) with regard to the size and shape of micelles. Both sides of the film are in contact with the solution. The arrows indicate alkali diffusion fluxes into pores. The film thickness is substantially larger than the pore diameter.
In order to clarify the question about the surfactant aggregation mechanism and its action on the track pore shapes, small angle neutron scattering experiments were performed with two different surfactants types: anionic (DSBS-Na) and nonionic nonylbenzenedecaethyleneoxide (NBDEO) [47]. Sodium hydroxide and potassium chloride were applied as electrolytes. The former is a component of etching solutions used to obtain nano- and micropores in ion-irradiated polymer films—in particular, polyethyleneterephthalate and polycarbonate films [39]. Since NaOH contains hydrogen atoms, causing enhanced incoherent scattering contributing to the background, additional measurements were performed with a system containing a neutral hydrogen-free electrolyte KCl.
The selected SANS curves, obtained for pure NBDEO and samples containing NBDEO with 1 M molar concentration of KCl and NaOH, are shown in figure 13. The changes in the curve slope at low q-region demonstrate the modifications of the shape of the micelles, which are formed upon addition of the surfactant. The analysis has shown that fitting using model objects characterized by an axial symmetry (cylinders, ellipsoids of revolution, etc) provides the best description of experimental data, consistent with the physical concepts of micelle shapes. The geometrical parameters of the micelles were finally evaluated using the cylindrical model.
Figure 13 Small-angle neutron scattering curves normalized with respect to surfactant concentration for NBDEO+1MKCl,NBDEO+1MNaOH and NBDEO samples.
Cylindrical micelles with a base radius equal to the surfactant molecule length were observed in experiments on both surfactants. The radius value, R M ≈1.5 nm, exhibits a weak dependence on surfactant concentration and remains about the same. The length of micelles is about L M ≈6.3 and 15.2 nm for pure NBDEO and DSBS-Na samples, respectively, and it increases upon addition of electrolytes. This effect is especially pronounced for NBDEO-based solutions, demonstrating the enlargement of micelle length by an order of magnitude, up to 163.2 nm.
The obtained experimental data allowed clarification of some features of track etching with surfactant-containing solutions. The enlargement of micelles with an increase in hydroxide concentration from 3 to 6 mol l −1 correlates with sharper (bullet-like) profiles of nanopores produced by track etching in a more concentrated alkali solution [44]. The modified mechanism for track formation during the chemical treatment of an ion-irradiated polymer with surfactant-containing etchant solutions is illustrated in figure 12(b). As the length of micelles is noticeably larger than the diameter of a track pore at an initial stage of its formation, micelle diffusion into pores is practically impossible, and the adsorbed layer may be formed inside pores only due to the diffusion of individual surfactant molecules. Hence, at the initial stages of etching, namely at the stage of the development of small (nanosized) pores, the process proceeds in the following way. Initially, the internal volume of a pore is inaccessible for both individual surfactant molecules and micelles. Thus, only sodium cations and hydroxide ions penetrate the pore. Hydroxide ions interact with the walls of the pore to widen it. Further, surfactant molecules become capable of diffusing into the pore, but micelles cannot yet penetrate the track channel. Individual surfactant molecules penetrating the channel are primarily located at the entry of the pore. Moreover, the average surfactant activity is substantially lower inside the pore than outside it, where its concentration is well above CMC [48].
The formation conditions of adsorbed layers inside the pore and outside it, i.e., on the flat polymer surface, are, most probably, equalized when the radius of the pore inlet markedly exceeds the micelle length. This assumption may explain the development of a cigar-like pore profile at an inlet size of more than 100 nm, when it exceeds the size of a surfactant molecule and the thickness of the adsorbed layer by an order of magnitude.
5. Summary
The reviewed results demonstrate that the application of neutron scattering methods provides important information about the structural organization and its relationship with physical properties of nanostructured materials and nanosystems. The information, obtained by neutron scattering methods, is essential for both fundamental and applied research in interdisciplinary fields of condensed matter physics, materials science, biology, medicine and pharmacology, as well as for the development of nanotechnologies.
Acknowledgments
The work has been supported by JINR-BRFFR Grant X10D-077 and State Contract 02.740.11.0542.